Beckman Function Generator Fg2 Manual High School
Tektronix Fg501a 2mhz Function Generator Module. Hp Agilent 53131a Universal Frequency Counter, 225mhz, Opt 010, Guaranteed Good. Hp Agilent E4433b Esg-d Signal Generator 250khz-4ghz, Tested Good W/options. Hp Hewlett Packard - 652a Test Oscillator 10 Mhz. Red Lion Controls Rpgc0061. Beckman Circuitmate Fg2 Function Generator Working. Customize your teaching materials in less time with Course Hero’s growing library of more than 10,000,000 lesson plans, study guides, and more. Gain access to Course Hero for free when you share your own resources with our community. Upload your content to help students and educators enhance their knowledge, too.
American Junior Academy of Sciences (AJAS) Poster Session
EXAMINING THE EFFICACY OF RNAI AS A POTENTIAL GENE THERAPY IN CAENORHABDITIS ELEGANS
The Cryopreservation of Aurelia aurita Polyps
NOSEMA SPECIES IN TRANSYLVANIA COUNTY: IMPLICATIONS FOR TREATMENT OF LOCAL HONEY BEES
The Role of hnRNPM in EMT and Breast Cancer Metastasis
SHINING LIGHT ON THE BLIND: EVOLUTIONARY REGRESSION & ADAPTIVE PROGRESSION IN R. BRAMINUS
THE EFFECT OF GABAPENTIN ON DUGESIA TIGRINA'S NERVOUS SYSTEM
THE EFFECT OF UV EXPOSURE ON CHELONIA MYDAS
DOES BETA-CELL SPECIFIC SPTLC2 DELETION IMPEDE DIABETIC ONSET?
OBSERVING THE EFFECTS OF ROUNDUP EXPOSURE ON THE ACTIVITY LEVELS OF C. ELEGANS
MIGRATORY BEHAVIOR AND HABITAT USE OF THE BULL SHARK IN THE SUB-TROPICAL WESTERN ATLANTIC
EFFECT OF MULTI-GENERATIONAL DEET EXPOSURE ON DROSOPHILA MELANOGASTER SENSITIVITY TO DEET
CHARACTERIZING ELECTROPHYSIOLOGICAL DATA RECORDED DURING BINGE EATING BEHAVIOR IN RATS
The Influences of the Zeigarnik Effect on Education
Using Caffeine and Taurine to Improve Memory Retention in Dugesia Dorotcephala
DISTINGUISHING PSYCHIATRIC SUBTYPES: BLINK RATE, SENSORY GATING, AND SYMPTOM DIFFERENCES
Shedding Light on Learning: The effect of ambient light on learning capability
The Effects of Toluene on Memory Retention in Planaria
INVESTIGATION OF WESTERN MODES: THE EFFECT OF A SCALE’S STARTING PITCH ON TONAL PERCEPTION
EFFICACY OF TOPOISOMERASE I SUMO-INHIBITING COMPOUNDS IN SENSITIZING CELLS TO CAMPTOTHECIN
NOVEL INHIBITORS OF GLUCOSE TRANSPORTER 1 FOR CANCER
FBXO-25’s Viability of a Prognostic Indicator of Effective Cancer Treatment
TOWARDS ACCURATE COPY NUMBER CALLING IN HIGH PLOIDY TUMORS
THE EFFECT OF MSI1 ON THE EPITHELIAL-MESENCHYMAL TRANSITION IN ADENOCARCINOMA CELLS
IL-10 INDUCES MACROPHAGE IMMUNOSUPPRESSION BY UPREGULATING TRANSCRIPTION FACTOR C-MAF
DEBUNKING DIETS: THE EFFECT OF HIGH-FAT DAIRY ON FERTILITY IN DROSOPHILA MELANOGASTER
The Effect of T-Bet Overexpression on Immune Factors In Vitro
DEVELOPMENT OF A REPORTER CONSTRUCT FOR RNA STABILITY MEASUREMENT IN HEK 293 CELLS
CCRCC CELLS WITH NF2 MUTATION RESPOND TO RECOMBINANT ERK AND AKT INHIBITOR TREATMENT
THE ROLE OF LIN-46, MOC-1, AND MOLYBDENUM COFACTOR IN C. ELEGANS DEVELOPMENT
INVESTIGATING THE ROLE OF TWO NEW GENES POTENTIALLY INVOLVED IN LIPID METABOLISM
IN SILICO DOCKING STUDIES OF CORDYCEPIN/PENTOSTATIN IN ADA1
Using Ab Initio Methods to Model the Structures of Disease-Causing ACVR1 Mutants
Enzymatic Hydrolysis: A Novel Approach to Direct Dye Removal
THE EFFECT OF ZEOLITE-GRAVEL MIXTURES ON THE CONCENTRATION OF LEACHATE
ENDOTOXIN-IMPRINTED POLYMERS: A NOVEL THERANOSTIC SOLUTION TO GRAM NEGATIVE SEPSIS
EFFECTIVENESS OF AMMONIUM NITRATE VS. UREA FOR USE IN INSTANT COLD PACKS
An Electrodynamic Approach to the Colligative Properties of Solutions
Extracting Biofuels from Spent Coffee Grounds and Waste Pine Needles
OXIDATION OF THIOL-ENE POLYMERS FOR THERMOMECHANICAL PROPERTY MODIFICATION
OPTICAL ENCRYPTION WITH CELLULOSE NANOCRYSTAL COMPOSITES FOR SECURITY APPLICATIONS
VerSign: Signature Identification and Verification using One Class Support Vector Machines
PREVENTATIVE ALGORITHMS TO CAPTURE THE EARLY ONSET OF CARBON MONOXIDE RELEASE
Project IRIS
ANALYSIS OF RRAM PERFORMANCE UNDER EXTREME TEMPERATURE CONDITIONS
AUTOMATED DIAGNOSIS OF DEMENTIA THROUGH SUPERVISED LEARNING CLASSIFICATION ALGORITHM
QUALITY-AWARE INCENTIVE MECHANISMS FOR MOBILE CROWDSENSING
A NOVEL SYSTEM FOR BRAIN TUMOR SEGMENTATION USING ARTIFICIAL NEURAL NETWORKS AND 3D SIFT
DETECTION OF THE EXTRASOLAR PLANET WASP-33B USING COLOR FILTER ANALYSIS
Quantifying the Intensity of Solar Flares by Sigmoid Pattern Analyses
ASSESSMENT OF SO2 POLLUTION IN CENTRAL PARK BY DNA BARCODING OF LICHEN SPECIMENS
DEVELOPING A NUMERICAL BOX MODEL TO COMPUTE ALGAE CONCENTRATION AS CHLOROPHYLL
Breathe Easy: Redesigning Inhalers for Asthma Patients
A DETERMINATION OF THE OPTIMAL SPEED OF A NOVEL OLEOPHILIC OIL SKIMMER
Communication by Ultrasound Using Radio Modulation Techniques
EXPLORING DESIGN POSSIBILITIES FOR ENHANCING DELIVERY EFFICIENCY OF A NEBULIZER
AN INTEGRATED DEVICE FOR SAMPLE PREPARATION, RNA AMPLIFICATION, AND DISEASE DIAGNOSTICS
AN ULTRASONIC DETECTION DEVICE FOR THE VISUALLY IMPAIRED
GAZ-EL TECH: AN INNOVATIVE METHOD TO REDUCE SUN GLARE FOR DRIVERS
PIEZO POWER: NONINVASIVE WIRELESS ENERGY TRANSFER FOR IMPLANTABLE MEDICAL DEVICES
THE EFFECTS OF NICKEL TITANIUM WIRE ON IMPACT FORCE
FABRICATION & EVALUATION OF DRY ELECTRODES IN A LONG-TERM WIRELESS HEART MONITORING SYSTEM
A SOLID CARBON DIOXIDE PRESSURE ENGINE FOR MARS COLONIZATION
Supercapacitor Electrode Derived from Polyacrylonitrile (PAN)/Poly(Styrene-co-acrylonitril
FROM ENVIRONMENTAL WASTE TO VALUE-ADDED PRODUCT
HOW GLYPHOSATE AND ROUNDUP® AFFECT THE GROWTH OF C. ELEGANS
BIOPLASTICS
Beckman Function Generator Fg2 Manual High School Denver
THE EXAMINATION OF HEAVY METALS LEACHING FROM FLY ASH CONCRETE
THE EFFECT OF MYCOREMEDIATION AND PHYTOREMEDIATION OIL SPILL COLLECTION ON WINTER RYE
EFFECTS OF COMPOST FROM ROUNDUP READY CORN STOVER ON THE CAENORHABDITIS ELEGANS
USE OF GRAPH THEORY TO DETERMINE EFFECT OF TAXONOMIC DIVERSITY LOSS ON TROPHIC FUNCTION
THE IMPACT OF ROUNDUP® ON DAPHNIA MAGNA
THE EFFICIENCY OF PASSIVE SOLAR HEATING, PART II
OIL SPILL CLEAN-UP: SORBENT EFFECTIVENESS NEAR AQUATIC SHORELINES
A Study Quantifying Stress-Induced Transcripts in Magnesium Bentonite Treated Bacteria
DETERMINING THE EFFECT OF PRETREATMENT ON THE YIELD OF CELLULOSIC ETHANOL FROM SWITCHGRASS
TOXICITY OF GLYPHOSATE ON THE C. ELEGANS WORM: IMPLICATIONS FOR SOIL AND HUMAN HEALTH
DETERMINING THE ECOTOXICITY OF TITANIUM DIOXIDE
DOSE RESPONSE OF D. MAGNA AND C. ELEGANS EXPOSED TO GLYPHOSATE
A Novel Approach to Purifying Greywater for Agricultural and Third World Applications
Can Tree Phenology Be Observed from 700 km Away in Space?
A Concrete Method for Recycling Printed Circuit Boards
THE EFFECT OF CONFECTIONERS SUGAR ON VARROA DESTRUCTOR
ANALYSIS OF THE ALGEBRAIC RELATIONSHIP OF POLYGON DIMENSIONS IN ORIGAMI SPIDER WEB DESIGN
DEVELOPING A FLIGHT PLAN TO REDUCE AIRCRAFT NOISE EXPOSURE IN CITIES
IDENTIFICATION OF MUTATED CANCER GENES THAT ARE SENSITIVE TO MITOCHONDRIAL DAMAGING DRUGS
NOVEL MATHEMATICAL MODEL TO PREDICT REPEATED ABLATION FOR RECURRENT ATRIAL FIBRILLATION
THE INTERPLAY BETWEEN THE BACK RX APP AND DISCOGENIC LOWER BACK PAIN
DESIGN AND EVALUATION OF BETULIN-BASED ANTI-CANCER COMPOUNDS
THE EFFECT OF CHLORINE AND BLEACH ON THE COLOR INTENSITY OF PIG SKIN
DEVELOPMENT OF A NOVEL TACROLIMUS PRODRUG TO TARGET THE COLON FOR TREATMENT OF COLITIS
UMBILICAL CORD TISSUE DERIVED MESENCHYMAL STEM CELLS: THERAPY FOR CYSTIC FIBROSIS
THE EFFECTIVENESS OF ANTIBACTERIAL MOUTHWASH
INVESTIGATING THE DESIGN OF GOLD NANOPARTICLES TO TARGET DIFFICULT-TO-REACH TUMOR SITES
TOXICOLOGICAL STUDIES ON THE EFFECTS OF CYPRIPEDIUM REGINAE ROOTS ON C. ELEGANS
WINDOW TO THE BRAIN: USING RETINAL BIOMARKERS TO DIAGNOSE ALZHEIMER'S DISE
THE EFFECTS OF NEAR INFRARED LIGHT AND CURCUMIN ON WOUND HEALING AND TISSUE REGENERATION
THE EFFECT OF DIFFERENT GENRES OF MUSIC ON BLOOD PRESSURE
THE EFFECT OF POLYPHENOLS IN SPICES ON THE AGGREGATION OF THE AMYLOID-BETA PEPTIDE 1-40
Enhancing Immunogenicity of Embryonic Stem Cells by Expression of GM-CSF
Combinatorial Effects of Bitter Melon and Temozolomide on Glioma Cells
TERMINATING CANCER: ENGINEERING THE TAXOL BIOSYNTHESIS PATHWAY IN E. COLI
OPTIMIZATION OF ANAEROBIC DIGESTION THROUGH THE INTRODUCTION OF RUMINANT METHANOGENS
REDUCING ANTIBIOTIC RESISTANCE AND MIC USING SILVER NANOPARTICLES WITH ANTIBIOTICS
ARMS-I A Novel Antiviral With Dual-Action Mechanism Against Influenza
DETECTING THE POSSIBLE ADULTERATION OF NON-GMO CORN AND SOY WITH GMO PRODUCTS
IDENTIFYING GENETICALLY MODIFIED ADULTERANTS IN OLIVE OIL USING PCR AND ELECTROPHORESIS
THE STUDY OF THE GENE(S) INVOLVED IN THE PROTECTION OF THE PSEUDOMONAS AERUGINOSA MUCOID S
ENTANGLING TIME-BIN QUBITS USING A SWITCH
IN VITRO BIOMINERALIZATION AND DEGRADATION OF MG DEGRADABLE IMPLANTS BY LASER PROCESSING
Phosphorene Nanodevice for Ultrafast Energy Harvesting and Next Generation Electronics
LEIA AND LOUISE: DATABASE DEVELOPMENT AND INTERFACING FOR ASTROPHYSICS RESEARCH
EXAMINING A NEW METHOD FOR THE DISCOVERY OF HYPOTHETICAL EXOTIC PARTICLES
ORIGINS OF THE LOW FRICTION EXHIBITED BY BIOCOMPATIBLE ZWITTERIONIC POLYMER BRUSHES
IRREGULAR MAGNET TOPOLOGIES IN AN ALTERNATING CURRENT (AC) GENERATOR
STEREOSCOPIC X-RAY RECONSTRUCTION ALGORITHMS FOR PREVENTING RADIATION INDUCED CANCER
EFFECTS OF HORTICULTURAL CHARCOAL ON THE CROP YIELD AND HEALTH OF EARLY GIRL TOMATOES
A NOVEL ENVIRONMENTALLY FRIENDLY METHOD FOR LIPID EXTRACTION FOR BIOFUEL PRODUCTION
PROGRESS IN MICROPROPAGATION OF CYPRIPEDIUM REGINAE
EFFECTS OF FREEZING CYPRIPEDIUM REGINAE SEEDS ON GERMINATION
Plant Talk: A Study of Interorganismic Communication Using Physical Adaptations of Phaseol
THE EFFECT OF AN ETHYLENE INHIBITOR ON THIGMOTROPISM IN TENDRILS
Abstract
We present a method that makes it possible to trigger, observe, and quantify membrane aggregation and fusion of giant liposomes in microfluidic chambers. Using electroformation from spin-coated films of lipids on transparent indium tin oxide electrodes, we formed two-dimensional networks of closely packed, surface-attached giant liposomes. We investigated the effects of fusogenic agents by simply flowing these molecules into the chambers and analyzing the resulting shape changes of more than 100 liposomes in parallel. We used this setup to quantify membrane fusion by several well-studied mechanisms, including fusion triggered by Ca2+, polyethylene glycol, and biospecific tethering. Directly observing many liposomes simultaneously proved particularly useful for studying fusion events in the presence of low concentrations of fusogenic agents, when fusion was rare and probabilistic. We applied this microfluidic fusion assay to investigate a novel 30-mer peptide derived from a recently identified human receptor protein, B5, that is important for membrane fusion during the entry of herpes simplex virus into host cells. This peptide triggered fusion of liposomes at an ∼6 times higher probability than control peptides and caused irreversible interactions between adjacent membranes; it was, however, less fusogenic than Ca2+ at comparable concentrations. Closely packed, surface-attached giant liposomes in microfluidic chambers offer a method to observe membrane aggregation and fusion in parallel without requiring the use of micromanipulators. This technique makes it possible to characterize rapidly novel fusogenic agents under well-defined conditions.
INTRODUCTION
The fusion of biological membranes is crucial for life (): viral fusion, exocytosis, organelle fusion, and fertilization of an oocyte are examples of biological processes that involve the merging of closed membranes. To study the molecular machinery and processes that govern the wide range of fusion events, cellular assays as well as assays in reconstituted systems can yield important insight and may ultimately lead to approaches to interfere with fusion processes for therapeutic purposes (). Here, we describe a versatile and practical fusion assay that proceeds in microfluidic chambers and makes it possible to investigate individual components of the fusion machinery under well-defined and variable conditions.
Membrane fusion typically involves three conserved steps: i), close contact between two membranes (3); ii), initial merging of the membranes, often associated with a hemifusion state (); and iii), opening of an aqueous fusion pore (). In living cells, membrane fusion is facilitated by proteins. Intracellular fusion of membranes involves the tethering of membranes by Rab proteins and the formation of SNARE (soluble N-ethylmaleimide sensitive factor attachment protein receptor) complexes to induce fusion (,). Fusion by viruses can be mediated by fusion peptides which are typically 15–30 amino acids long. When activated, these peptides insert into the target membrane and trigger fusion (,). The regulation of the interactions between proteins, lipids, and other molecules in these cellular processes is complex. Artificial lipid membranes, in contrast, offer systems for studying individual parameters involved in membrane fusion and for determining minimal models of this process (–). Weber et al. showed that complementary SNARE proteins reconstituted into separate liposomes were sufficient to induce fusion (). Other studies employing artificial vesicles have shown that a range of external factors affect fusion, including mechanical stress (–), divalent and trivalent cations (–), long-chain polymers (,), high-strength electric fields (), and membrane curvature (–).
Several techniques have been developed to investigate membrane fusion in artificial systems. Fusion assays typically assess the mixing of lipids and of the contents of liposomes when fusogenic agents are added. These measurements usually employ small liposomes (typically <200 nm) with high radii of curvature and utilize fluorescence techniques (–), light scattering (), or electron microscopy (,) for detection. In these assays, the size of liposomes affects fusion; recently, Nomura et al. reported that the hemagglutinin (HA) fusion peptide promoted fusion of liposomes with diameters <200 nm but did not induce fusion of giant liposomes ().
Fusion assays using cell-sized, giant liposomes allow microscopic observation of membrane fusion and assessments of mixing of lipids and contents. Such experiments typically use micropipette techniques to bring two liposomes into close contact (,). For this approach, liposomes adhere to a micropipette through suction (), and micromanipulators position the micropipettes to establish contact between two liposomes (–). Other methods have utilized electrophoresis to fuse oppositely charged liposomes (), dielectrophoresis to bring liposomes together for electrofusion (), or a mixing chamber (). Here we present a method that affords fusion assays with the following five characteristics: i), it enables direct visualization of membrane aggregation and fusion of cell-sized liposomes in a microfluidic chamber; ii), it monitors hundreds of membrane-membrane contacts in parallel; iii), it allows controlled triggering of fusion by biologically relevant mechanisms under physiological conditions without the need for manual control; iv), it investigates fusion of lipid membranes of well-defined composition in the absence or presence of fusogenic agents; and v), it provides the opportunity to vary the composition of the aqueous environment in which the fusion process occurs.
In previous work, Chiu et al. trapped two cells or liposomes in a microfluidic system (by optical trapping, dielectrophoresis, or micromanipulation) and used high-strength electric fields (E > 100 kV·m−1) to fuse the cells (–). We adopted a different approach to obtain giant liposomes in close contact by drawing upon techniques recently developed in our lab: we formed densely packed networks of surface-attached giant liposomes in flow chambers (,). These liposome networks allowed the introduction of fusogenic molecules to the liposomes in the flow chambers and provided a means to visualize fusion events directly in an optically transparent setup. Unlike most fusion assays with giant liposomes, this setup made it possible to observe many membrane-membrane interactions in parallel. Moreover, it required no micromanipulators to bring liposomes into close contact. We validated this microfluidic fusion assay by triggering fusion with well-studied fusogenic agents such as calcium ions and polyethylene glycol (PEG). We then reconstituted a minimal model of protein-mediated fusion that was based on biospecific interaction between membranes. Finally, we demonstrate the usefulness of this technology by performing a fusion assay on a recently characterized peptide that is involved in the entry of herpes simplex virus (HSV) into host cells. The results show that triggering membrane fusion in microfluidic chambers under well-defined conditions can yield important insight into the fusogenic properties of novel molecules.
MATERIALS AND METHODS
Preparation of a microfluidic setup for fusion assays
The microfluidic setup used in this work consisted of four parallel flow chambers in which we formed surface-attached giant liposomes (Fig. 1). Detailed methods for fabrication have been described previously (). Briefly, we embedded four pieces of silicone tubing (inside diameter = 0.60 mm, Dow Corning, Midland, MI) in a slab of 2.1-mm-thick poly(dimethylsiloxane) (PDMS, Sylgard 184 Silicone, Dow Corning) () and extracted four rectangular chambers (29.1 mm × 3.24 mm × 2.22 mm, volume = 209 μL) using a surgical blade. This PDMS structure was sandwiched between two large aluminosilicate glass slides (50 mm × 50 mm × 1.1 mm) covered each with a surface of indium tinoxide (ITO, R, = 5-15 ohms. Delta Technologies, Stillwater, MN). The assembly was held together by binder clips (Officemate International, Edison, NJ) (Fig. 1). Before assembly of the setup, we prepared films of lipids by spin coating (). Solutions of 3.75 mg mL−1 lipids (mixtures of L-α-phosphatidylcholine (egg, chicken) (eggPC), 1-palmitoyl-2-oleoyl-sn-glycero-3-phosphocholine (POPC), 1-palmitoyl-2-oleoyl-sn-glycero-[phospho-rac-(1-glycerol)] (POPG), 1-palmitoyl-2-oleoyl-sn-glycero-3-phosphoethanolamine (POPE), and 1,2-dipalmitoyl-sn-glycero-3-phosphoethanolamine-N-(cap piotinyl) (sodium salt) (N-cap biotin-PE) all from Avanti Polar Lipids, Alabaster, AL) in 95% chloroform (Acros Organics, Morris Plains, NJ), 5% acetonitrile (Acros Organics, Geel, Belgium) were spin coated onto ITO at a speed of 600 rpm for 100 s. Films of lipid were dried under vacuum (−740 mTorr) for 2 h to remove traces of solvent. We used the same PDMS flow chambers in all experiments with the wash protocols for the PDMS flow chambers and the plates of ITO as described previously (,).
Microfluidic setup for forming surface-attached giant liposomes and triggering fusion of these liposomes. A PDMS spacer with embedded silicone tubing leading into and out of four parallel flow chambers was sandwiched between two glass plates each covered with a surface of ITO. Films of lipids were spin coated onto the surfaces of ITO before assembly of the setup. Giant liposomes were formed by electroformation after filling the chambers with solution. An AC electric field was applied to the ITO surfaces over 2 h to form liposomes. This setup was optically transparent and allowed the introduction of new solutions to the chambers at defined flow rates using a microprocessor-controlled syringe pump.
Formation of giant liposomes in microfluidic chambers
We employed electroformation, a technique developed by Angelova and Dimitrov, to form giant liposomes (48,49). Application of alternating current (AC) voltages to electrodes with films of lipids on their surface induces the swelling of giant, unilamellar liposomes from the lipid film (50,). We hydrated the spin-coated film of lipids in the flow chambers by filling, in succession, each chamber with solution while applying an AC voltage of 1.6 V peak-to-peak (Vpp) at a frequency of 10 Hz using a function generator (Circuitmate FG2, Beckman Coulter, Fullerton, CA) attached to the ITO electrodes. We allowed electroformation to proceed for 2 h before turning off the electric field.
Flow procedures for triggering fusion
To replace solutions inside the flow chambers, we used a programmable syringe pump (KD Scientific, Holliston, MA) to drive fluid flow at volumetric flow rates below 5.0 mL h−1. For experiments involving Ca2+-induced fusion, we formed giant liposomes from 90% POPC, 10% POPE in solutions of 0.1 mM Tris (hydroxymethyl) aminomethane (Tris, Shelton Scientific, Shelton, CT) buffer pH 7.4. We then introduced 0–100 μM solutions of CaCl2 (EMD Chemicals, Gibbstown, NJ) that contained 0.1 mM Tris buffer at a rate of 2.6 mL h−1 to induce fusion.
To examine two-dimensional aggregation of giant liposomes, we formed giant liposomes composed of 100% eggPC in solutions of glycerol that were isoosmolar to the CaCl2 to be introduced to the chamber. Solutions of CaCl2 (ranging from 0.1 μM to 200 mM) were flowed through the chamber of liposomes for 1 h at rates <4.0 mL h−1.
For experiments with poly-ethylene glycol (PEG), we employed giant liposomes composed of 90% POPC, 10% POPE. To examine PEG-induced fusion with osmotic mismatches between the inside and outside of liposomes, we formed giant liposomes in 0.1 mM Tris buffer pH 7.4 and introduced 1, 5, and 10 mM PEG-6000 (Calbiochem, San Diego, CA) at 2.0 mL h−1 for 1 h. We also performed experiments with osmotically matched solutions of PEG-6000. Using an osmometer (Model 3320, Advanced Instruments, Norwood, MA) to match osmolalities, we formed giant liposomes in solutions of 1, 10, and 38 mM sucrose (EM Industries, Gibbstown, NJ) and then introduced to the chambers 1, 5, and 10 mM PEG-6000, respectively (see Fig. S3 for osmolality concentration curves for PEG-6000 and sucrose). Finally, to compare PEG-6000 to the other fusogenic molecules used in this assay, we introduced 25 μM PEG-6000 in 0.01× phosphate buffered saline (PBS) to giant liposomes prepared exactly as described below for experiments with the fusion peptide.
For experiments involving “receptor-mediated” fusion, we formed giant liposomes composed of 95% POPC, 5% biotinyl-PE in deionized H2O (diH2O). We introduced 1.67 μM NeutrAvidin biotin-binding protein (Molecular Probes, Eugene, OR) dissolved in diH2O at a rate of 2.5 mL h−1 to induce fusion.
Fusion assay with peptide from B5 protein
We performed a fusion assay with a synthetic peptide from the C-terminus of the B5 receptor protein that can facilitate entry of HSV into host cells (). This 30-amino acid peptide (denoted WT-B5 peptide), corresponded to amino acids 344–374 (KQQWQQLYDTLNAWKQNLNKVKNSLLSLSD) of the B5 protein. It was synthesized and purified to 98% by the University of Michigan protein core facility (). The peptide was acetylated at the N-terminus, amidated at the C-terminus, and dissolved in PBS to a concentration of 2.5 mM. We used two other peptides as control peptides in the fusion assay, the oxidized chain B of insulin from bovine pancreas (Sigma, St. Louis, MO) and human adrenocorticotropic hormone (ACTH) residues 1–24 (AnaSpec, San Jose, CA), dissolved in diH2O without further modification.
For all fusion assays with WT-B5 peptide, giant liposomes composed of 90% POPC, 10% POPE were formed in 0.1 mM Tris buffer pH 7.4. We prepared solutions of WT-B5 peptide by diluting the stock solutions (2.5 mM WT-B5 peptide in PBS) in diH2O. In the case of oxidized chain B of insulin and ACTH (–), we added PBS to the diluted solutions to match the osmolarity and ionic strength of the solutions of WT-B5 peptide at a given concentration of peptide. Before introducing the solutions of peptides to the liposomes, we flowed solutions of PBS through the chamber of liposomes at 2.5 mL h−1 for 1 h to replace the Tris buffer inside and outside of the surface-attached liposomes; we demonstrated previously that new solutions introduced to surface-attached giant liposomes replace the previous solution both outside and inside the liposomes (). These solutions were isoosmolar to the solution of peptide to be introduced, thus ensuring that osmolarity differences were not a factor in the fusion assay. After filling the chambers with PBS, we introduced the peptide solutions to the chambers at a rate of 2.5 mL h−1 for 1 h for all experiments.
Observation and quantification of fusion
We observed giant liposomes and membrane fusion by phase contrast microscopy using an inverted microscope (Eclipse TE 2000-U, Nikon, Melville, NY) with 10× and 20× objectives with extra long working distance in phase-contrast mode. We captured images and movies of liposomes using a charge-coupled device camera (Photometrics CoolSnap HQ, Roper Scientific, Trenton, NJ). Image analysis software (Metamorph from Universal Imaging Corporation, Downington, PA) allowed for determination of the size of liposomes. Fusion events were observable and could be quantified by visually examining movies frame-by-frame for the occurrence of merging of liposomes (see Supplementary Material for movies).
In the fusion assay for the WT-B5 peptide, precautions were taken to ensure that similar experimental conditions (with respect to the number, average diameter, and curvature of liposomes in the field of view) were used (see Supplementary Material, Fig. S4). In each experiment, we chose regions of initially spherical liposomes (consisting of 100–120 closely packed liposomes). We acquired movies of each peptide solution that was introduced to the liposomes. For analyses, we visually counted the number of fusion events (a fusion event was defined as two liposomes merging into a new liposome with a larger diameter than each of the initial two liposomes), as well as the time of the event and the diameter of liposomes that fused. We defined the metric “probability of fusion” as the number of liposomes that fused divided by the total number of liposomes in the field of view; we used this value to compare results between fusion experiments.
To quantify two-dimensional aggregation of giant liposomes (e.g., in the case of Ca2+-induced aggregation), we developed an image-processing algorithm using the PERL language (see Supplementary Material for detailed discussion of algorithm). This image-processing program analyzed phase-contrast micrographs of surface-attached giant liposomes and returned the value of “percentage of shared membrane” for the image. This metric was defined as the average percentage of each liposome that was directly in contact with adjacent liposomes.
RESULTS AND DISCUSSION
Formation of networks of surface-attached giant liposomes in microfluidic chambers
We formed cell-sized giant liposomes in transparent flow chambers by electroformation from spin-coated films of lipids on ITO electrodes (). By growing liposomes from uniform films of lipids with optimal thickness, we obtained densely packed networks of giant liposomes (in which most liposomes were in close contact with several neighbors) across the entire surfaces of formation (Fig. 2 A) (). We did not detach the liposomes; detachment is typically performed by applying a low frequency AC electric field (53). Instead, the giant liposomes remained affixed to the film of lipids on the surface of ITO by lipid tethers () even when exposed to flow. This experimental approach made it possible to monitor, in parallel, many membrane-membrane contacts between giant liposomes using a phase-contrast microscope. Additionally, we were able to observe the same region of liposomes upon introduction of new solutions to the flow chambers to follow directly the effect of the introduced molecules on membrane-membrane interactions and fusion. We used this setup in all subsequent fusion assays.
Triggering fusion by introducing fusogenic molecules to surface-attached giant liposomes. (A) Ca2+-induced fusion: the introduction of 25 μM CaCl2 promoted the fusion of 17% liposomes (composed of 90% POPC, 10% POPE) in the field of view. The arrows show the merging liposomes before introduction of Ca2+ (left column) and after 260 s of flow with a flow rate of 2.6 mL h−1 (right column). (B) Fusion triggered by a hydrophilic polymer: Flowing 10 mM PEG-6000 into the chamber induced the fusion of liposomes made of 90% POPC, 10% POPE. (C) Fusion triggered by biospecific interactions: Liposomes containing 95% POPC, 5% biotin-PE fused when 1.67 μM neutravidin was introduced to the chamber of liposomes. Initially spherical liposomes underwent several fusion events to form a single giant liposome (right column shows an intermediate stage in the fusion process). Scale bars = 50 μm. See Supplementary Material for complete movies of these processes.
Ca2+-induced fusion of giant liposomes
The fluidic setup presented here made it possible to rapidly investigate several fusogenic agents by simply flowing in solutions of the molecules and analyzing the resulting shape changes of the liposomes. To test the microfluidic fusion assay on a well-known process, we introduced Ca2+ ions to giant liposomes in the flow chamber. Calcium ions bind to the phosphate group of phospholipids, thus reducing charges at the surface of liposomes and promoting aggregations (–). In addition, Ca2+ can bridge the headgroups of adjacent vesicles and promote fusion through the creation of “defects” in neighboring bilayers (). The formation of these defects, and hence fusion, is stochastic (,). When we introduced 25 μM CaCl2 to giant liposomes, 17% of all liposomes in the field of view (total 164 with an average diameter of 30.6 ± 11.5 μm) fused within 1 h (see Supplementary Movie S1 for this Ca2+-induced fusion). Most fusion events (85%) occurred in the first 8 min of flow (Fig. 2). In comparison, introducing 5 μM CaCl2 triggered fusion of only 1.6% of liposomes (observing 128 liposomes with an average diameter of 33.4 ± 10.0 μm). These low probabilities of fusion highlight an advantage of directly examining many membrane-membrane interactions in parallel (,).
Two-dimensional aggregation of giant liposomes
Membrane fusion is preceded by close contact between adjacent membranes. Therefore, the tendency of membranes to aggregate in the absence or presence of “membrane-active” molecules is important to evaluate membrane-membrane interactions and fusion. We found that after introducing 25 μM CaCl2 to giant liposomes and after the concomitant fusion of 17% of the liposomes, ∼15% of liposomes underwent “two-dimensional aggregation” (Fig. 2 A, right hand side). In the context of this work, we defined two-dimensional aggregation as the changes in shape of surface-attached giant liposomes to increase contacts with neighboring liposomes (with all the liposomes imaged in the same two-dimensional plane, Fig. 2). These increased interactions had three characteristics: 1), the number of vesicle-vesicle contacts between liposomes that were initially not in contact increased (Supplementary Material, Fig. S1B); 2), vesicles adhered to one another (Supplementary Material, Fig. S1B); and 3), contact points between liposomes extended into defined lines (Fig. 3, C and E).
Quantification of Ca2+-induced two-dimensional aggregation of surface-attached giant liposomes composed of 100% eggPC. (A) An image processing algorithm processed the micrographs of giant liposomes after 1 h of flow of CaCl2 to determine the “percentage of shared membrane”, which represents the average percentage of each liposome that is in contact with the visible membrane of adjacent liposomes (N = 3 for all points; error bars represent standard deviations). Images (B–E) show representative phase-contrast micrographs of two-dimensional aggregation after introducing solutions of (B) H2O, (C) 500 μM CaCl2, (D) 10 mM CaCl2, and (E) 200 mM CaCl2 to the liposomes. Note that the liposomes did not exhibit two-dimensional aggregation after introducing an intermediate concentration of 10 mM CaCl2. Scale bars = 75 μm.
To examine this two-dimensional aggregation, we quantified the interactions between surface-attached giant liposomes in solutions of 0–200 mM CaCl2 (Fig. 3). We developed an image processing algorithm to compute “percentage of shared membrane”, which represents the average percentage of each visible liposome membrane in contact with adjacent liposome membranes (see Supplementary Material for a full discussion of image processing techniques). This quantification showed that giant liposomes exhibited significant two-dimensional aggregation between 100 μM and 1 mM CaCl2 and also at CaCl2 concentrations higher than 200 mM (Fig. 3 A). Interestingly, liposomes did not aggregate at an intermediate concentration of 10 mM CaCl2 (Fig. 3 D), presumably due to binding of sufficient Ca2+ to the headgroups of lipids to induce positive-charge repulsion between bilayers ().
By quantifying interactions between giant liposomes, we were able to compare this two-dimensional assay to other three-dimensional techniques. Previously, Akashi et al. monitored the adhesion of pairs of giant liposomes composed of zwitterionic lipids in solutions of CaCl2 (). Liposomes adhered at concentrations of CaCl2 between 50 μM to 1 mM and also at concentrations above 30 mM. Liposomes did not adhere at concentrations below 10 μM, and they also did not adhere at concentrations between 1 and 30 mM (). Marra and Israelachvili obtained similar results using a direct force measuring apparatus on adsorbed planar lipid bilayers (). Therefore, the results presented here for two-dimensional aggregation are in agreement with previous measurements of adhesion energies from Akashi et al. and from Marra and Israelachvili. With respect to comparing fusion in the two-dimensional case versus three-dimensional spectroscopic techniques, however, it is difficult to compare different fusion assays because several parameters (e.g., sizes of liposomes, composition of lipids) can drastically affect fusion (,). Most spectroscopic assays employ small and large unilamellar vesicles (SUVs and LUVs, respectively), which have significantly smaller sizes and higher curvature than the giant liposomes used here. Wilschut et al. suggested, however, that the tendency of vesicles to fuse after aggregation induced by Ca2+ is related to the tendency of vesicles to aggregate (). Since aggregation was comparable between the assay presented here and previously reported three-dimensional techniques and since the presented assay shows that increasing the concentration of fusogenic agents such as Ca2+ increased the fusion probability, we suggest that the microfluidic two-dimensional fusion assay can deliver results that are at least qualitatively comparable to established fusion assays.
PEG-induced fusion: effects of osmotic mismatches
In addition to Ca2+-induced fusion, we tested the assay with PEG. PEG is a hydrophilic polymer that has been employed to induce fusion of both cells and artificial vesicles (). The well-known mechanism for PEG-triggered fusion derives from two effects: i), mechanical stress induced by the differences in osmolarity across the membranes of liposomes (); and ii), dehydration of bilayers by PEG (). In previous spectroscopic assays studying PEG-induced fusion, osmotic mismatches have been used to enhance fusion, as negative osmotic pressure (when the solution inside liposomes is hypotonic compared to the solution around liposomes, normally associated with shrinkage of liposomes) promoted fusion of LUVs in the presence of PEG ().
Using the assay presented here, we first examined fusion of surface-attached giant liposomes after introducing osmotically unmatched solutions of PEG-6000 (at concentrations of 25 μM, 1 mM, 5 mM, 10 mM PEG-6000). Both 5 mM and 10 mM PEG induced massive fusion of giant liposomes (Fig. 2 B). In the case of 10 mM PEG, the liposomes appeared brighter than the surrounding PEG solution within 20 s after introducing 10 mM PEG to surface-attached liposomes; this difference was a result of mismatches in index of refraction of the PEG solution surrounding liposomes and the hypotonic solution inside the liposomes (Fig. 2 B, left-hand side) (). Immediately after this gray-scale shift, liposomes fused massively with adjacent vesicles (Fig. 2 B, right-hand side). Concomitantly, the interactions between membranes increased strongly, and after 75 s, the majority (>75%) of the liposomes in the field of view had fused (some of these liposomes subsequently ruptured; see Supplementary Movie S2 for the full video of fusion). In contrast to the massive fusion triggered by 5 mM and 10 mM PEG, 25 μM PEG (a concentration that typically is far too low to trigger PEG-induced fusion) did not result in fusion or aggregation of giant liposomes ().
In the case of 1 mM PEG-6000, however, giant liposomes shrank upon introduction of PEG. This shrinkage occurred on a timescale of minutes, and it was complete after ∼20 min. Liposomes did not fuse, and we did not observe increased contacts between giant liposomes. Instead, the giant liposomes decreased their size such that only a few of them retained points of contact with neighboring giant liposomes. We attribute the absence of noticeable shrinkage of liposomes after introducing 5 and 10 mM PEG to the rapid fusion at these elevated concentrations. Presumably, in these cases, fusion occurred before significant shrinkage (due to osmotic mismatches) could occur.
We also examined PEG-induced fusion of giant liposomes using osmotically matched solutions of 1 mM, 5 mM, and 10 mM PEG-6000 (by forming liposomes in solutions of sucrose isoosmolal to the PEG solution to be introduced, see Supplementary Material, Fig. S3). With osmotically matched solutions, we observed that 5 mM and 10 mM PEG induced rapid fusion of giant liposomes. As expected, with osmotically matched 1 mM PEG, liposomes did not shrink. Instead, the liposomes experienced two-dimensional aggregation; we did not observe any fusion events within the field of view with 1 mM PEG.
Osmotic mismatches, therefore, can affect the results from the fusion assay presented here. To explore further the effects of osmotic mismatches by themselves, we created osmotic gradients (up to 5 mOsm/kg) with sucrose, a small nonfusogenic molecule. After introducing 5 mM sucrose to giant liposomes formed in 0.1 mM Tris, we did not observe any fusion events or aggregation. Most importantly, surface-attached giant liposomes did not shrink. Similarly, after reintroduction of 0.1 mM Tris to the chamber, the liposomes did not fuse or aggregate.
We attribute the absence of shrinkage or fusion of liposomes in the case of 5 mM sucrose to the presence of tubular openings that connect surface-attached giant liposomes to the film of lipids on the surface of the ITO electrode (,53). Previously we showed that small solutes (including ions, sucrose, and water) exchanged rapidly through these tubules into (and out of) surface-attached liposomes; larger molecules (>3 kDa) did not exchange readily through these tubules (). Therefore, as long as we were exchanging low molecular weight molecules (e.g., Ca2+ or sucrose) we observed only small, transient osmotic mismatches, as the tubular opening of the liposomes both allowed small molecules to enter liposomes and possibly also relieved osmotic pressure, thereby minimizing osmotic mismatches. Introducing 1 mM PEG-6000, however, did generate mismatches that were significant enough to shrink giant liposomes because the PEG did not exchange rapidly through the lipid tubules.
This example with PEG-6000 highlights several important aspects of the fusion assay presented here. First, when introducing macromolecules (>3 kDa), it is important to match osmolarities (unless the effects of osmotic mismatches are intended). For small molecular weight compounds (e.g., ions and sucrose) small mismatches in osmolarities do not cause shrinkage or swelling of vesicles because of lipid tubules that allow rapid diffusion into surface-attached liposomes. Second, to analyze aggregation and fusion, it is critically important to establish and maintain sufficient contacts between liposomes while introducing solutions to the flow chambers. Since the giant liposomes are surface attached in a two-dimensional matrix, they have limited lateral mobility to establish contacts with neighboring liposomes. Liposomes can either remain as they are, change their shape (e.g., by two-dimensional aggregation with neighbors), fuse, or shrink. Therefore, shrinkage of liposomes or examining fields of view with sparsely populated liposomes might generate nonrepresentative results since aggregation and fusion cannot occur if liposomes are spaced too far apart. For the fusion assays presented here, we always observed densely packed regions of giant liposomes (Fig. S4), and we only observed shrinkage of liposomes in the one case of introducing an unmatched solution of 1 mM PEG-6000. Despite these considerations, the presented aggregation and fusion assays are typically straightforward to perform since electroformation of giant liposomes from spin-coated films of lipids typically results in a dense two-dimensional network of surface-attached liposomes (,) and since matching osmolarities circumvents the problem of liposome shrinkage.
Fusion triggered by biospecific interactions
We utilized the strong binding interactions between biotin and neutravidin for triggering fusion and thus tested the microfluidic fusion assay on a reconstituted, minimal model of protein-mediated fusion. We formed surface-attached liposomes containing a biotinylated lipid and flowed a solution of 1.67 μM neutravidin in deionized water into the chamber (Fig. 2 C). Liposomes started to fuse immediately with their neighbors. This lateral fusion proceeded over 25 min until most liposomes (∼80%) in the field of view had merged into one, large liposome with a diameter >600 μm (see Supplementary Movie S3 for a full movie of this fusion process). No lateral fusion of membranes occurred in a control experiment in which neutravidin was introduced to liposomes that did not contain biotin-PE.
The mechanism for this fusion process stems from biospecific interactions between adjacent membranes. Neutravidin has four binding sites with high affinity for biotin (–). When introduced to liposomes containing biotin-PE lipids, neutravidin presumably bound between adjacent liposomes, thus bringing the membranes into close contact. Once in contact, the membranes fused possibly due to the dehydration of membranes or from formation of defects by a large number of bridging molecules; several research groups showed previously that such defects can promote fusion (–). This neutravidin-driven mechanism may be considered as a minimal model of protein-mediated fusion in cells, such as fusion mechanisms based on SNARE complexes (,). Liposome fusion by biotin-neutravidin interactions also complements studies of fusion of liposomes with complementary DNA on the headgroups of their lipids (). The microfluidic fusion assay presented here makes it straightforward to investigate such minimalist models of biological fusion events, while providing the opportunity to increase the complexity of the model by introducing additional molecules.
Fusion assay for the WT-B5 peptide, which is involved in viral entry of herpes simplex virus
To demonstrate the usefulness of the presented microfluidic setup for characterizing novel, biologically relevant fusion processes, we performed a fusion assay with a 30-amino acid peptide (WT-B5 peptide) from the C-terminus of B5, a cell-surface membrane receptor protein that enables viral entry of HSV into host cells (,). The presence of synthetic WT-B5 peptide blocks HSV infection in human and other B5-expressing cells by competitive inhibition (). Moreover, Perez et al. showed that high concentrations (∼42 mM) of WT-B5 peptide was capable of fusing adjacent cells even in the absence of proteins from HSV (). Here, we investigated the fusogenic activity of WT-B5 peptide on adjacent giant liposomes. This assay had the benefit that it proceeded under well-defined conditions of, e.g., membrane composition, buffer composition, and peptide concentration; we hypothesized, therefore, that it may make it possible to elucidate the contributions of individual molecules to the overall fusion event during viral entry of HSV.
Beckman Function Generator Fg2 Manual High School System
We introduced WT-B5 peptide at concentrations between 0.5 and 25.0 μM to surface-attached giant liposomes. WT-B5 peptide induced fusion of giant liposomes, with a probability of fusion that was concentration dependent (Fig. 4 A and Supporting Movie S4). Maximal fusion occurred at 10 μM WT-B5 peptide, where the probability of fusion was 9.7%. Although a fusion probability below 10% was moderate, two-dimensional aggregation of membranes in the presence of the peptide occurred for all liposomes (Fig. 4 B). Even at 0.5 μM WT-B5 peptide, a concentration at which fusion was very rare, we observed significantly increased membrane-membrane interactions.
Fusion assay for WT-B5 peptide compared with control peptides using giant liposomes composed of 90% POPC, 10% POPE. (A) We measured the average probability of fusion (defined as the number of liposomes that fused divided by the total number of liposomes in the field of view) for different concentrations of (▪) WT-B5 peptide, (•) ACTH (–), and (▴) the oxidized chain B of insulin. For WT-B5 peptide, the fusion probability was concentration dependent and enhanced compared to the control peptides. (B) The introduction of 5-μM WT-B5 peptide to initially spherical liposomes triggered the fusion of up to 10% of liposomes. The panels in B show a close-up of one of the fusion events at t = 30 s followed by increased interactions of membranes and two-dimensional aggregation over the course of the next 770 s. Scale bar = 40 μm.
The rate of this two-dimensional liposome aggregation depended on the concentration of WT-B5 peptide. At 25 μM WT-B5 peptide, most (75%) of membranes deformed within 200 s across the entire field of view; at 0.5 μM, liposomes interacted gradually over the course of 1 h (flow rate for all concentrations: 2.6 mL h−1). Interestingly, this aggregation was not reversible by flowing isoosmolar solutions of PBS through the chamber over the course of 2 h. This result indicated that a strong interaction occurs between WT-B5 peptide and adjacent liposome membranes composed of 90% POPC and 10% POPE.
We compared these results with fusion induced by two other short peptides with lengths and physicochemical properties similar to WT-B5 peptide (Table 1): ACTH (–); and the oxidized chain B of insulin (30 amino acids). The two control peptides each contained predicted regions of helix formation (similar to WT-B5 peptide, Table 1) (–). Utilizing the same experimental conditions as in studies of WT-B5 peptide, we found that these two peptides rarely triggered fusion of membranes (Fig. 4). We did, however, observe increased interactions between giant liposomes after introducing 5 μM concentrations of both the oxidized chain B of insulin and ACTH (–). ACTH (–) caused rapid deformation of membranes, and insulin chain B produced a more gradual interaction II than ACTH. Interestingly, this two-dimensional aggregation was reversible for both 5 μM ACTH (–) and 5 μM insulin chain B by flowing isoosmolar solutions of PBS through the chambers to flow out the peptide solutions. This result contrasts with irreversible membrane aggregation with 5 μM WT-B5 peptide under the same conditions, as mentioned above.
TABLE 1
Sequence and structural properties of the peptides employed in fusion assay
Name of peptide | Sequence | Charge * | Average index of hydrophobicity * | Predicted structure in H2O |
---|---|---|---|---|
WT-B5 peptide | KQQWQQLYDTLNAWKQN-LNKVKNSLLSLSD | +2 | −2.7 | Predicted α-helix and coiled-coil formation (52,74) |
Oxidized chain B of insulin | FVNQHLCoxGSHLVEALYL-VCoxGERGFFYTPKA | 0 | −0.8 | Central α-helix (9–17) with well-defined β-turn (17–21) (76) |
ACTH (1–24) | SYSMEHFRWGKPVGKKR-RPVKVYP | +6 | −2.7 | Hydrophobic regions (1–10) partition into POPC membrane (with potential helical structure), charged regions (11–24) associate with membrane surface (75) |
The results of this fusion assay for WT-B5 peptide suggest several characteristics about the interaction of this peptide with membranes. First, WT-B5 peptide interacts strongly with membranes of zwitterionic phospholipids such as PC and PE, possibly by partial insertion into these membranes. Second, membrane aggregation due to WT-B5 peptide was irreversible within 2 h, whereas this two-dimensional aggregation was reversible when induced by control peptides. Previous studies have indicated that other fusion peptides, such as HA, insert into bilayers and disrupt the packing of lipids (,). The observation that WT-B5 peptide induced fusion at a significantly higher probability than the control peptides supports the hypothesis that WT-B5 peptide inserts into membranes, whereas the control peptides appeared to associate peripherally or insert weakly into the membranes of liposomes (–).
A second implication of the results with WT-B5 peptide, which has been documented for other fusion peptides and molecules, is that aggregation of membranes induced by WT-B5 peptide is not sufficient to induce a high probability of fusion (). Although almost all membranes aggregated at concentrations of WT-B5 peptide above 1 μM, the probabilities of fusion remained below 10%. Both control peptides also induced the two-dimensional aggregation of membranes but did not trigger fusion. Presumably, aggregation was due to dehydration of membranes combined with neutralization of surface charges on liposomes (from negatively charged impurities in PC lipids which are usually present) (55,), and the formation of fusogenic defects was rare.
Finally, the results from this assay suggest that WT-B5 peptide by itself does not constitute the complete machinery necessary for effective viral fusion of HSV. Although WT-B5 peptide was successful in inducing some fusion of giant liposomes, these events were not as efficient as examples involving Ca2+ or biospecific tethering. Although the probabilities of fusion for 25 μM WT-B5 peptide were higher than for 25 μM PEG-6000, they were significantly lower than the probabilities for 25 μM Ca2+ or 1.67 μM neutravidin. It is likely that the addition of viral ligands or host cell proteins to the system would increase the probabilities of fusion. We believe that the fluidic assay presented here will be useful for the step-by-step reconstruction of parts of the fusion machinery of a range of fusion processes.
CONCLUSIONS
The capability to form closely packed giant liposomes that are attached to the surface of a flow chamber made it possible to develop a versatile and practical fusion assay. In this assay, possible fusogenic agents could be introduced into the chamber, and the fusogenic effects of these molecules could be observed on many membrane-membrane contacts in parallel. In addition, the microfluidic setup allowed exchanging solutions inside the chambers, leading to replacement of solutions both outside and inside surface-attached giant liposomes (). This exchange of solutions allowed controlling the microenvironment around liposomes, making it possible to investigate membrane aggregation and fusion in a range of solutions with varying properties such as ionic strength (), pH, or the presence of soluble molecules, peptides, proteins, etc.
Using this assay, we demonstrated that microfluidic assays of liposome fusion can reveal quantitative information on the probability of membrane fusion triggered by four different stimuli, including i), divalent cation-induced aggregation and destabilization of membranes; ii), dehydration of membranes by PEG; iii), receptor-mediated interactions between membranes; and iv), fusion promoted by a peptide involved in viral entry of HSV. Visualization of membrane fusion provided at least four advantages for studying membrane fusion. First, visualization was advantageous for studying the changes in shape of individual liposomes before fusion (e.g., two-dimensional aggregation, Fig. 3). Second, the fusion assay presented here provided information about the individual size of each liposome that fused, as well as the time after introduction of the fusogenic agent. Third, using surface-attached giant liposomes may allow monitoring changes in the shapes of liposomes during the fusion process. In a recent article, Lei and MacDonald used high-speed microfluorescence spectroscopy to study intermediate lipid-mixing structures in the fusion of giant liposomes (). It is conceivable that high-speed phase-contrast microscopy techniques could reveal useful information about the changes in shape of giant liposomes in response to introduced fusogenic agents before and during fusion, using the assay presented here. And fourth, being able to observe the fusion process provided information about the mechanism of fusion events. In the case of the WT-B5 peptide, the concentration-dependent, irreversible two-dimensional aggregation of membranes indicated that this peptide interacts strongly with and possibly partitions into bilayers. This interaction was sufficient to induce fusion of giant liposomes but not to the extent to conclude that WT-B5 peptide by itself is a highly efficient fusion peptide.
Despite these numerous advantages, several aspects of this assay could be further improved. The current methods that we used for quantifying fusion required detecting and measuring fusion events visually, and this analysis can be time consuming. We believe, however, that image processing algorithms can be developed (perhaps similar to the algorithms we present in the Supplementary Material to quantify two-dimensional aggregation) to detect fusion in an automated procedure to simplify and accelerate the analysis. Also, the assay in its present form does not reveal quantitative information on lipid and contents mixing, two parameters that can provide kinetic information in spectroscopic assays (). Therefore, existing spectroscopic techniques may currently offer rapid means to quantify fusion, whereas the assay presented here enables direct visualization of fusion events or aggregation of cell-sized giant vesicles. We believe that triggering and observing membrane fusion in microfluidic chambers provides a broadly applicable and straightforward procedure for performing fusion assays on cell-sized liposomes under well-defined and variable conditions.
SUPPLEMENTARY MATERIAL
An online supplement to this article can be found by visiting BJ Online at http://www.biophysj.org.
Acknowledgments

We thank Amy P. Wong for her insightful suggestions, Shuichi Takayama for use of the spin-coater, and Jodi L. Liu for her careful measurements.
This work was supported by the National Science Foundation (02-111 CAREER Award, M.M.). D.J.E. acknowledges a National Institutes of Health Cellular Biotechnology Training Program (CBTP) fellowship and a Rackham Graduate Fellowship at the University of Michigan. S.R.L. acknowledges the Genetics Training Program and a Rackham graduate fellowship at the University of Michigan.
References
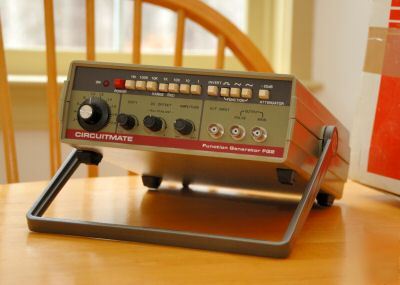
